Shunts are defined in terms of the ratio of pulmonary blood flow (Qp) to systemic blood flow (Qs). In normal haemodynamics, the two flows are equal: Qp = Qs. In L-to-R shunts, the Qp/Qs ratio is > 1. If this reaches > 1.5-2, surgery is indicated, as the pulmonary blood flow is twice the systemic blood flow. In R-to-L shunts, the Qp/Qs ratio is < 1. A ratio of 0.5:1 indicates that the pulmonary blood flow is half the systemic blood flow. Therapy is indicated for all R-to-L shunts. The pulmonary blood flow may be increased (L-to-R shunt) or reduced (R-to-L shunt, pulmonary stenosis). A long-term increase in pulmonary blood flow can lead to pulmonary arterial hypertension (PAH), while reduction of pulmonary blood flow leads to cyanosis.
Shunts exhibit four main characteristics: flow direction (L-to-R, R-to-L or bidirectional), orifice size (restrictive or non-restrictive), downstream cavity dilation, and the changes they cause in terms of pulmonary blood flow [2]. A shunt’s blood flow is determined by the diameter of the orifice (or duct), pressure in the upstream cavity, and flow resistance in the downstream cavity. The flow is systolic and diastolic with two peaks in velocity, which normally do not exceed 1 to 4 m/s [1]. Independently from its dimensions, the flow of a shunt increases if the upstream pressure rises or the downstream pressure drops.
Shunts exhibit four main characteristics: flow direction (L-to-R, R-to-L or bidirectional), orifice size (restrictive or non-restrictive), downstream cavity dilation, and the changes they cause in terms of pulmonary blood flow [2]. A shunt’s blood flow is determined by the diameter of the orifice (or duct), pressure in the upstream cavity, and flow resistance in the downstream cavity. The flow is systolic and diastolic with two peaks in velocity, which normally do not exceed 1 to 4 m/s [1]. Independently from its dimensions, the flow of a shunt increases if the upstream pressure rises or the downstream pressure drops.
- Left → right shunt (anomalous pulmonary venous return, ASD, AV canal, VSD, ductus arteriosus) (Figure 14.8) (Table 14.4): it is reduced if SVR falls or PVR rises. In ASDs, it is increased if LAP rises due to a fall in LV compliance (diastolic dysfunction). High SaO2 levels do not necessarily indicate that O2 transport (DO2) is satisfactory. When the Qp/Qs ratio ≥ 3:1, DO2 falls even if the arterial blood is sufficiently oxygenated (risk of cyanosis). Pulmonary circulation overload leads relatively quickly to pulmonary hypertension, congestive ventricular failure, and pulmonary vascular disease with a PVR so high that it secondarily leads to a bidirectional shunt (Eisenmenger's syndrome).
Figure 14.8: Left → right shunt. It is increased if SVR rises and PVR falls, in which case pulmonary blood flow (Qp) is excessive. The shunt is reduced if SVR falls and PVR rises.
- Right → left cyanotic shunt (tetralogy of Fallot, VSD + pulmonary atresia, transposition of the great arteries, single ventricle) (Figure 14.9) (Table 14.5): the shunt is increased if SVR falls or PVR rises. Cyanosis is proportional to the drop in systemic pressure. To reduce a cyanotic shunt, pulmonary resistance and right-sided afterload must be lowered or systemic resistance and left-sided afterload increased. SaO2 fluctuates in line with the Qp/Qs ratio. An increase in FiO2 has a negligible effect on cyanosis linked to a R-to-L shunt as this is caused by blood flow that does not cross the lungs. SaO2 ≥ 75% is generally well tolerated, but surgery is indicated if the value is < 70-75%, as this corresponds to Qp/Qs < 0.5:1.
Figure 14.9: Right → left shunt. It is increased when SVR falls, which lowers the pulmonary arterial blood flow (Qp). The shunt is reduced if SVR increases and PVR falls. As a result, pulmonary flow increases and SaO2 improves.
- Left → right palliative shunt (Blalock-Taussig shunt, central aortopulmonary shunt, aortopulmonary collaterals) (Figure 14.10): blood flow is determined by the diameter of the shunt or collaterals. It results in left ventricular overload. The pulmonary blood flow is directly proportional to the systemic pressure and PVR: SaO2 decreases if SVR falls (hypotension, hypovolemia) or if PVR rises. Balance is sometimes difficult to achieve. Although α-stimulants (phenylephrine, noradrenaline) slow down arterial desaturation, their risk is to increase significantly the Qp/Qs ratio and to prompt pulmonary overload and systemic hypoperfusion. In most cases, it is better to adjust PVR (low FiO2, moderate hypoventilation). Systemic diastolic pressure is low due to constant leakage in the pulmonary vascular bed, which may compromise coronary circulation.
- Bidirectional shunt: if the orifice is wide and the pressure gradient low, blood may flow in one direction during systole and in the other during diastole, or may vary according to SVR, PVR, and respiratory pressure. While O2 saturation in the pulmonary artery is higher than in the systemic venous return, saturation in the aorta is lower than in the pulmonary veins. In a bidirectional shunt, the effective flow is the component of the total flow in the aorta and pulmonary artery that originates from the appropriate circulation, where total flow (Qt) is the sum of effective flow (Qeff) and flow recirculated by the shunt (Qrecirc) [3]. In cases of transposition of the great arteries, where the two circulations run in parallel and not in series, the effective flow is actually the shunt flow, which occurs via the ASD or VSD (see Transposition of the great arteries). In the physiology of a single ventricle or truncus arteriosus, the two circulations are interdependent. Since PVR is lower than SVR, the pulmonary circulation tends to “steal” blood from the systemic circuit, especially during diastole. Overloading of the pulmonary artery is accompanied by systemic hypoperfusion (myocardial ischaemia) and metabolic acidosis.
Figure 14.10: Left → right corrective shunt to increase pulmonary blood flow (Qp) in case of pulmonary stenosis. Its blood flow diminishes as SVR falls and also rises as SVR increases.
If the defect is small, the shunt is described as restrictive since its flow rate is low and the pressure gradient is high. Flow through the orifice is more or less fixed and minimally affected by changes in the ratio between pulmonary and systemic vascular resistance (PVR/SVR). This type of shunt keeps a stable haemodynamic significance, varying little during anaesthesia and surgery. Examples include small VSDs. If the defect is large, the shunt is described as non-restrictive, with little gradient or even equalisation of pressures through the communication. The haemodynamic significance of this type of shunt is dependent on the PVR/SVR ratio. In extreme cases, the defect is so large that the two structures form a single common chamber (single atrium, single ventricle). In general, the larger the shunt, the more its blood flow is dependent on the ratio between PVR and SVR. Shunts in the ventricles (VSD) and arteries (ductus arteriosus, Blalock shunt) are dependent on the PVR/SVR ratio, while shunts in the atria (ASD) are more dependent on the respective compliance of the right and left cavities.
In general, the shunt causes the receiving cavity to dilate and overloads the upstream ventricle. L-to-R shunts in the ventricles (VSD) or arteries (ductus arteriosus, Blalock shunt) increase pulmonary blood flow and therefore venous return to the left side of the heart. They primarily cause volume overload of the LV, which is the pump driving blood flow through the shunt. This flow mainly occurs during systole when the pulmonary valve is open. The RV operates as a passive conduit. Only the gradual increase in PVR causes a secondary pressure overload of the RV. In contrast, a L-to-R shunt situated upstream of the atrioventricular valves (ASD, anomalous pulmonary venous return) creates a volume overload for the RV, which must pump the increased volume into the pulmonary artery (Figure 14.11).
The primary goal in the management of children with shunts is to balance the pulmonary blood flow (Qp) and systemic blood flow (Qs), and achieve a Qp/Qs ratio close to 1:1. To that end, PVR and SVR are adjusted to lower Qp in an L-R shunt (high PVR and low SVR) and increase Qp in an R-to-L cyanotic shunt (low PVR and high SVR). In the first instance, FiO2 is lowered and PaCO2 increased through hypoventilation to induce respiratory acidosis (pulmonary vasoconstriction). Haematocrit may also be raised to help increase viscosity. In contrast, in the second instance, FiO2 is increased and PaCO2 is lowered through hyperventilation to induce alkalosis. In the case of an R-to-L cyanotic shunt, the procedure is not aimed at achieving the highest possible SaO2, but at balancing the two circuits: Qp must ensure satisfactory SaO2 (often 75-85%) while Qs must ensure normal supply to the tissues (with no metabolic acidosis). The Qp/Qs ratio must be as close as possible to 1:1. Shunts potentiate the effects of hypovolemia on arterial pressure due to the constant loss of volume in the low-pressure pulmonary circuit.
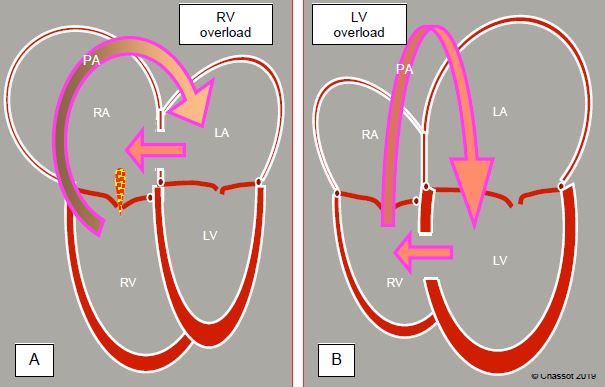
Figure 14.11: Ventricular overload due to an L → R shunt. A: In pre-tricuspid shunts (ASD, anomalous pulmonary venous return), the RA and RV are dilated (cavities downstream of the shunt). After the pulmonary artery, the blood returns to the LA and flows back through the RA. The volume overload is taken on by the RV, which is dilated and hyperdynamic. The apex of the heart is formed by the RV and not the LV. This volume overload is well tolerated for 2-3 decades. B: In post-tricuspid shunts (VSD, ductus arteriosus) the blood pumped by the LV flows straight through the pulmonary artery and returns to the LA and LV via the pulmonary veins. Volume overload occurs in the LV. However, this type of shunt causes both pressure and volume overload. This is less well tolerated and the right ventricle hypertrophies when the pulmonary pressure reaches high levels.
These data can be mathematically quantified using two laws of physics adapted to haemodynamics:
In general, the shunt causes the receiving cavity to dilate and overloads the upstream ventricle. L-to-R shunts in the ventricles (VSD) or arteries (ductus arteriosus, Blalock shunt) increase pulmonary blood flow and therefore venous return to the left side of the heart. They primarily cause volume overload of the LV, which is the pump driving blood flow through the shunt. This flow mainly occurs during systole when the pulmonary valve is open. The RV operates as a passive conduit. Only the gradual increase in PVR causes a secondary pressure overload of the RV. In contrast, a L-to-R shunt situated upstream of the atrioventricular valves (ASD, anomalous pulmonary venous return) creates a volume overload for the RV, which must pump the increased volume into the pulmonary artery (Figure 14.11).
The primary goal in the management of children with shunts is to balance the pulmonary blood flow (Qp) and systemic blood flow (Qs), and achieve a Qp/Qs ratio close to 1:1. To that end, PVR and SVR are adjusted to lower Qp in an L-R shunt (high PVR and low SVR) and increase Qp in an R-to-L cyanotic shunt (low PVR and high SVR). In the first instance, FiO2 is lowered and PaCO2 increased through hypoventilation to induce respiratory acidosis (pulmonary vasoconstriction). Haematocrit may also be raised to help increase viscosity. In contrast, in the second instance, FiO2 is increased and PaCO2 is lowered through hyperventilation to induce alkalosis. In the case of an R-to-L cyanotic shunt, the procedure is not aimed at achieving the highest possible SaO2, but at balancing the two circuits: Qp must ensure satisfactory SaO2 (often 75-85%) while Qs must ensure normal supply to the tissues (with no metabolic acidosis). The Qp/Qs ratio must be as close as possible to 1:1. Shunts potentiate the effects of hypovolemia on arterial pressure due to the constant loss of volume in the low-pressure pulmonary circuit.
Figure 14.11: Ventricular overload due to an L → R shunt. A: In pre-tricuspid shunts (ASD, anomalous pulmonary venous return), the RA and RV are dilated (cavities downstream of the shunt). After the pulmonary artery, the blood returns to the LA and flows back through the RA. The volume overload is taken on by the RV, which is dilated and hyperdynamic. The apex of the heart is formed by the RV and not the LV. This volume overload is well tolerated for 2-3 decades. B: In post-tricuspid shunts (VSD, ductus arteriosus) the blood pumped by the LV flows straight through the pulmonary artery and returns to the LA and LV via the pulmonary veins. Volume overload occurs in the LV. However, this type of shunt causes both pressure and volume overload. This is less well tolerated and the right ventricle hypertrophies when the pulmonary pressure reaches high levels.
These data can be mathematically quantified using two laws of physics adapted to haemodynamics:
- Ohm's Law: Q = P / R (cardiac output = pressure / resistance)
- Poiseuille’s Law: Q = (ΔP • r4) / 8 • η • L
where: P = pressure
r = pipe (shunt) radius
η = blood viscosity
L = pipe length
A shunt's blood flow is therefore proportional to the pressure gradient through the orifice and to the fourth power of the radius of this orifice. It is inversely proportional to resistance through the shunt and to the shunt's length.
The shunt is clearly demonstrated with echocardiography by colour flow and spectral Doppler, and also by direct visual inspection during surgery. A shunt's value (Qp/Qs) can be calculated using echocardiography based on the ratio of the flow or systolic volume through the pulmonary artery and through the aorta (or the systemic outflow tract). A shunt may be more reliably calculated by catheterisation, by determining the O2 enrichment of the pulmonary artery or the O2 depletion of the aorta using Fick's principle [2]:
r = pipe (shunt) radius
η = blood viscosity
L = pipe length
A shunt's blood flow is therefore proportional to the pressure gradient through the orifice and to the fourth power of the radius of this orifice. It is inversely proportional to resistance through the shunt and to the shunt's length.
The shunt is clearly demonstrated with echocardiography by colour flow and spectral Doppler, and also by direct visual inspection during surgery. A shunt's value (Qp/Qs) can be calculated using echocardiography based on the ratio of the flow or systolic volume through the pulmonary artery and through the aorta (or the systemic outflow tract). A shunt may be more reliably calculated by catheterisation, by determining the O2 enrichment of the pulmonary artery or the O2 depletion of the aorta using Fick's principle [2]:
- Pulmonary blood flow (Qp) = VO2 / (CvpO2 - CapO2)
- Systemic blood flow (Qs) = VO2 / (CaoO2 - CvO2)
- Shunt (Qp / Qs) = (CaoO2 - CvO2) / (CpvO2 - CapO2)
where: VO2 = O2 consumption
CaoO2 = O2 content in the aorta artery
CvO2 = central venous O2 content
CvpO2 = pulmonary venous O2 content (≈ CaoO2)
CapO2 = O2 content in the pulmonary artery
Since pulmonary venous O2 content is very similar to that observed in the aorta, it can be substituted by CaoO2. Arterial O2 content (CaO2) is the total of O2 carried by Hb and of dissolved O2:
CaO2 = Hb (g/L) x 1.34 (mL O2/g Hb) x SaO2 + PO2 (mmHg) x 0.003 (mL O2/mmHg/dL)
Since measurements are taken at room air (FiO2 0.21), dissolved O2 is negligible and may be deleted. Since the quantity of Hb is the same in all simultaneous samples, it is possible to use O2 saturation only. Therefore, when calculating the shunt, saturations can be used instead of O2 content. This changes the formula to:
Shunt (Qp / Qs) = (SaO2 - SvO2) / (SaO2 - SapO2)
Sampling for saturations should be performed upstream and downstream of the shunt. O2 saturation of the RA is the mean value of three separate blood flows: the SVC accounts for 30% of venous return in adults (40-50% in infants) with an SvO2 value of 75-77%, while the IVC accounts for 65% of venous return with an SvO2 value of 78%, and the coronary sinus accounts for 5% of return with 25% saturation. RA and pulmonary artery saturation are therefore normally: 0.30 x 77% + 0.65 x 78% + 0.05 x 25% = 75%. A mean saturation value of 96% in ambient air is observed for both the LA and pulmonary veins [3]. Thermodilution with a pulmonary artery catheter is used to measure the pulmonary blood flow (Qp), while PiCCO systems are used to measure systemic blood flow (Qs). These values are not equal if a shunt is present. On the other hand, the recirculation of cold liquid through an ASD or VSD leads to an underestimation of the actual flow by the Swan-Ganz catheter.
CaoO2 = O2 content in the aorta artery
CvO2 = central venous O2 content
CvpO2 = pulmonary venous O2 content (≈ CaoO2)
CapO2 = O2 content in the pulmonary artery
Since pulmonary venous O2 content is very similar to that observed in the aorta, it can be substituted by CaoO2. Arterial O2 content (CaO2) is the total of O2 carried by Hb and of dissolved O2:
CaO2 = Hb (g/L) x 1.34 (mL O2/g Hb) x SaO2 + PO2 (mmHg) x 0.003 (mL O2/mmHg/dL)
Since measurements are taken at room air (FiO2 0.21), dissolved O2 is negligible and may be deleted. Since the quantity of Hb is the same in all simultaneous samples, it is possible to use O2 saturation only. Therefore, when calculating the shunt, saturations can be used instead of O2 content. This changes the formula to:
Shunt (Qp / Qs) = (SaO2 - SvO2) / (SaO2 - SapO2)
Sampling for saturations should be performed upstream and downstream of the shunt. O2 saturation of the RA is the mean value of three separate blood flows: the SVC accounts for 30% of venous return in adults (40-50% in infants) with an SvO2 value of 75-77%, while the IVC accounts for 65% of venous return with an SvO2 value of 78%, and the coronary sinus accounts for 5% of return with 25% saturation. RA and pulmonary artery saturation are therefore normally: 0.30 x 77% + 0.65 x 78% + 0.05 x 25% = 75%. A mean saturation value of 96% in ambient air is observed for both the LA and pulmonary veins [3]. Thermodilution with a pulmonary artery catheter is used to measure the pulmonary blood flow (Qp), while PiCCO systems are used to measure systemic blood flow (Qs). These values are not equal if a shunt is present. On the other hand, the recirculation of cold liquid through an ASD or VSD leads to an underestimation of the actual flow by the Swan-Ganz catheter.
Shunts |
A shunt has 4 characteristics
- Its direction (L-to-R non-cyanotic, R-to-L cyanotic) - Its size (restrictive or non-restrictive) - The dilation of the receiving chambers - The increase or decrease of the pulmonary blood flow A shunt located upstream of the atrioventricular valves causes a volume overload for the RV, while a shunt in the ventricular or arterial area causes a volume overload for the LV. The chronic rise in pulmonary blood flow gradually increases the PAP, secondarily causing pulmonary hypertension and a pressure overload for the RV. The shunt is defined by its relationship to systemic flow: Qp/Qs. Treatment is aimed at bringing this Qp/Qs ration as close as possible to 1:1 (Qp = Qs). The aim with cyanotic R-to-L shunts is not to obtain the highest possible SaO2, but rather to balance the two circuits: the Qp must ensure satisfactory SaO2 (often 75-85%) whille the Qs must ensure normal supply to the tissues (absence of metabolic acidosis). |
© BETTEX D, BOEGLI Y, CHASSOT PG, June 2008, last update February 2020
References
- BETTEX D, CHASSOT PG. Transesophageal echocardiography in congenital heart disease. In: BISSONNETTE B, edit. Pediatric anesthesia. Basic principles, State of the art, Future. Shelton (CO): People’s Medical Publishing House (USA), 2011, 1186-1212
- DUPUIS C, KACHANER J, PAYOT M. Cardiologie pédiatrique. Paris: Flammarion, 1991, 137-42
- JOFFE DC, SHI MR, WELKER CC. Understanding cardiac shunts. Pediatric Anaesth 2018; 28:316-25